The Little Furnace That Could: Using Space to Improve Radiation Detection
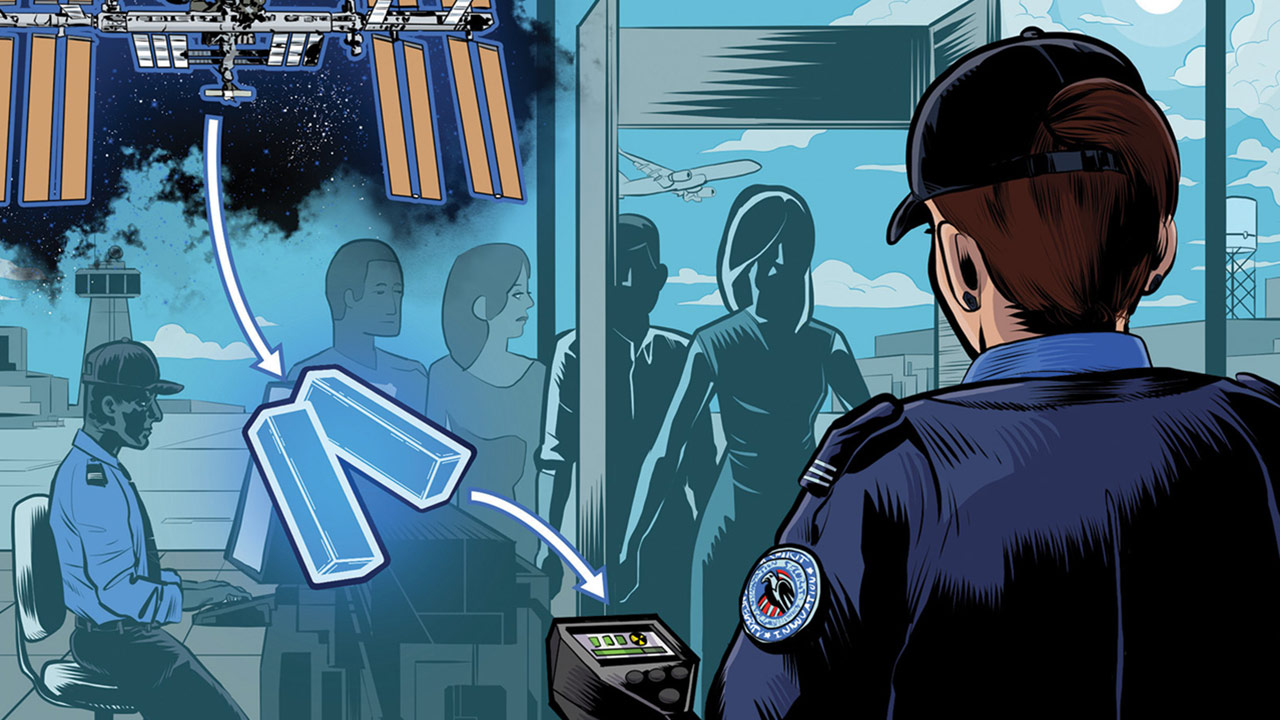
November 27, 2017 • By Roberto Molar-Candanosa, Contributing Author
Everything around you emits radiation—from the bananas in your kitchen, to cosmic rays from space, to the piece of paper (or screen) from which you are reading this sentence. Some of these types of radiation are so insignificant that they are harmless. But some can be dangerous. So, how can we better distinguish and detect different types of radiation?
One way is to improve the semiconductor and scintillator crystals used as radiation detectors. Two investigations that launched to the ISSInternational Space Station National Lab in April are studying such crystals using the recently refurbished Solidification Using a Baffle in Sealed Ampoules (SUBSA) hardware. A high-temperature furnace roughly the size of a small microwave oven, SUBSA can be used to study how microgravityThe condition of perceived weightlessness created when an object is in free fall, for example when an object is in orbital motion. Microgravity alters many observable phenomena within the physical and life sciences, allowing scientists to study things in ways not possible on Earth. The International Space Station provides access to a persistent microgravity environment. influences the synthesis of these crystals, which could ultimately enable improved functionality on Earth.
GROWING CRYSTALS ON EARTH
Electronic devices—including computers, medical imagers, and radiation detectors—function thanks to semiconductors: small and specially produced crystals used to conduct electrical signals. Smaller and more powerful semiconductors are the reason our pocket-sized cellphones are so smart these days.
Conversely, scintillators are unique crystals that emit light when exposed to certain types of energy, much like glow-in-the-dark objects. They are mostly used in radiation detection devices for homeland security and defense applications, X-ray security cameras, and medical imaging devices.
To create both semiconductors and scintillators, scientists combine and melt various elements that crystalize after cooling down. The functionality of these crystals depends not only on their composition, but also on their purity and quality and the physical conditions they are exposed to during cooling.
Producing these high-quality crystals is not easy, and many difficulties in the production process can be blamed on gravity. Earth’s gravity drives convection, the movement within and between fluids with different temperatures or densities, causing hot fluids to rise and cold or dense fluids to sink while transferring heat.
Aleksandar Ostrogorsky, Illinois Institute of Technology professor and principal investigator of one of the current SUBSA crystal growth projects, said that when crystals are grown on Earth using slow and precise solidification techniques, gravity-driven convection is a problem. It results in variable melt composition, which disrupts the radiation detection properties of the grown crystals.
“There’s a continuing mixing in the melt when you grow crystals, as there is when you are boiling a pot of tea,” Ostrogorsky said.
These complex dynamics lead to crystals with problematic heterogeneous composition, cracks, bubbles, and other structural imperfections, which disrupt the performance of the crystal in downstream applications.
For example, to activate crystals with radiation detection capabilities, small quantities of a substance known as a dopant must be distributed uniformly throughout the crystal. On Earth, convection causes continuous and unpredictable movement as the melt crystallizes, disturbing dopant distribution.
For many years, scientists have thus aimed to achieve diffusion-controlled growth to grow crystals without the complexities caused by convection. That’s where the SUBSA facility on the ISS National Lab could provide a competitive advantage. In space, buoyancy forces are virtually eliminated in the near-absence of gravity. This reduced motion allows for a more homogeneous distribution of dopants, fewer imperfections during crystallization, and ultimately, better crystals.
THE FIRST SUBSA EXPERIMENTS
In 2002, the SUBSA furnace was launched to space to study semiconductors made of indium antimonide. A specially designed transparent section of the SUBSA furnace allowed for observation and video-recording of the melting and crystallization processes onboard the ISS.
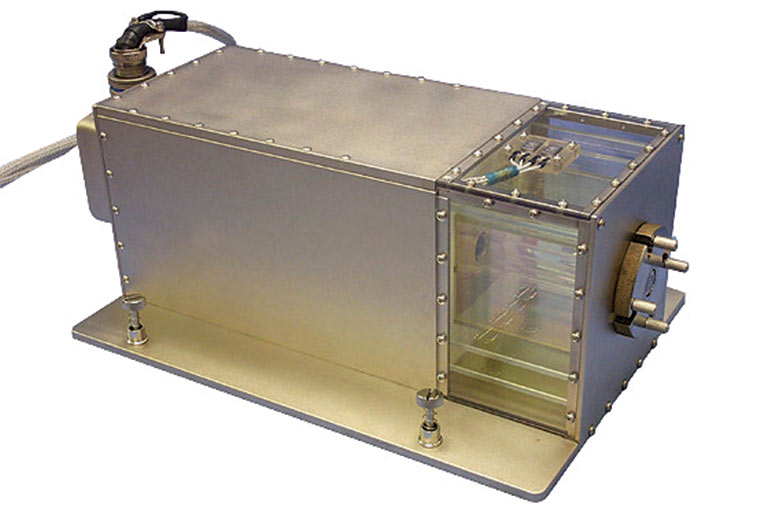
Media Credit: Tec-Masters, Inc./NASA
During Ostrogorsky’s first SUBSA experiments in 2002, melting and directional solidification was observed for the first time under microgravity conditions. A data downlink from the video-enabled furnace on the ISS allowed Ostrogorsky to coordinate with NASANational Aeronautics and Space Administration Astronaut Peggy Whitson to change temperatures, melt times, and other factors, observing how they affected crystallization. These experiments facilitated better understanding of the complex effects of convection in crystallization.
The 2002 successes laid the foundation for a new era of SUBSA utilization kicked off by Ostrogorsky and his former student, Alexei Churilov, who is now a senior scientist at Radiation Monitoring Devices, Inc. (RMD), in Watertown, Massachusetts (see A New and Improved SUBSA Furnace). In this round, Ostrogorsky is examining a different semiconductor material that is safe and affordable to produce, and Churilov is examining a scintillator crystal with the powerful ability to distinguish between various types of damaging radiation.
GROWING SEMICONDUCTOR CRYSTALS IN SPACE
The new generation of SUBSA payloads seeks to meet growing technological demands of the U.S. Department of Homeland Security and the U.S. Department of Energy, both of which supported Ostrogorsky’s research in the gap period between NASA-sponsored SUBSA experiments. Ostrogorsky’s present SUBSA investigation on the ISS National Lab will test indium iodide, which is chemically similar to the indium antimonide samples he used in 2002.
“Indium iodide is an overall promising detector for nuclear radiation,” Ostrogorsky said, “and it is the only one I know that is completely benign, meaning it doesn’t contain poisonous elements.”
Indium iodide can be prepared inexpensively and has a low melting point of 365°C. “That’s a relatively easy job for the little SUBSA furnace,” Ostrogorsky said. It is also simple to synthesize and reacts to gamma rays, making it suitable for radiation detection.
Ostrogorsky said that his current ISS National Lab experiment, launched on the Orbital ATK CRS-7 in April, will help determine the theoretical limit of the crystal’s detection capabilities and other benchmark properties of semiconductors. The experiment is examining de-wetting, which prevents stress-induced imperfections, via separation of the melt from the crystal growth container—a process that has never been directly observed and that Ostrogorsky will watch thanks to the video-enabled SUBSA.
“If at the end this gives us a very good reference for high-quality materials, then we’ll have a specific goal to continue this on Earth,” Ostrogorsky said. “We could get a really good detector if we manage to control these properties.”
SYNTHESIZING SCINTILLATORS IN MICROGRAVITY
Although semiconductors are a powerful tool for identifying harmful gamma radiation, they cannot detect neutrons, an important type of radiation emitted from objects such as nuclear bombs. However, Cs2LiYCl6:Ce (also known as CLYC) scintillator crystals, another set of samples being tested inside the refurbished SUBSA furnace, are capable of detecting this critical signature of nuclear weapons.
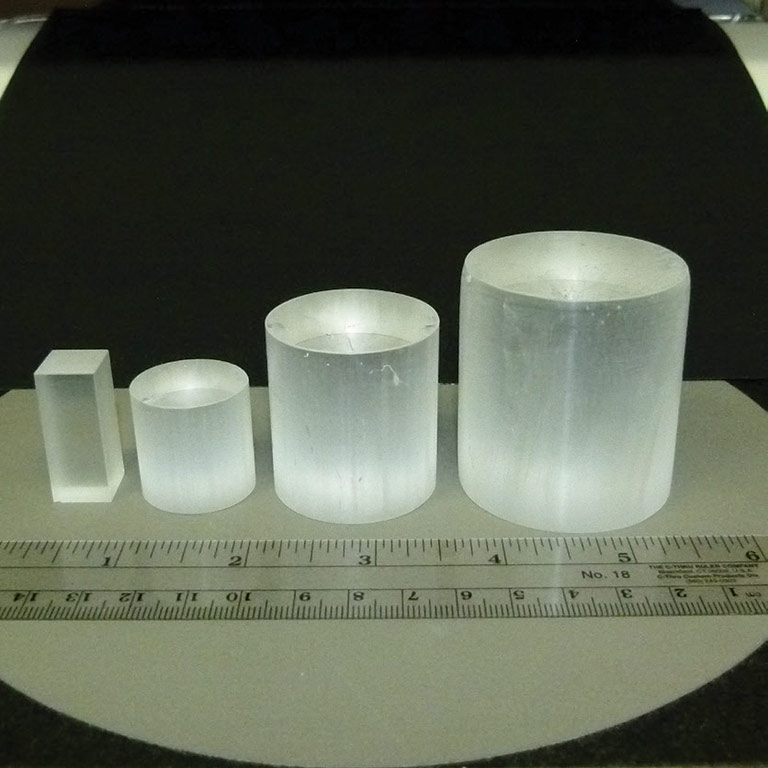
CLYC scintillator crystals
Media Credit: Radiation Monitoring Devices, Inc.
Because neutrons do not normally interact with other materials, they are more difficult to detect. The ability to detect neutron radiation is the distinctive quality of CLYC scintillator crystals, said Churilov.
Developed by RMD with support from the U.S. Defense Threat Reduction Agency and Domestic Nuclear Detection Office, CLYC scintillator crystals can detect both gamma rays and neutrons. Furthermore, CLYC scintillators can differentiate between types of radiation. Such dual detection is useful for many applications. For example, CLYC scintillators could help minimize the number and types of detection devices needed for airport security personnel.
“Using one instrument with CLYC scintillators, you could distinguish between harmless sources of radiation versus something like cesium-137 for a dirty bomb,” Churilov said. Current technology to detect neutrons is limited, so CLYC scintillator crystals could be game-changers in the field of radiation detection.
To move toward more widespread use of CLYC scintillator crystals for radiation detection devices, RMD is now focusing on scaling up production to grow crystals with larger diameters and fewer physical defects. “But when you scale up the size during crystal growth, defects are much more important,” Churilov said. “Crystals crack more easily, they can grow bubbles in them, and so it’s much more difficult.”
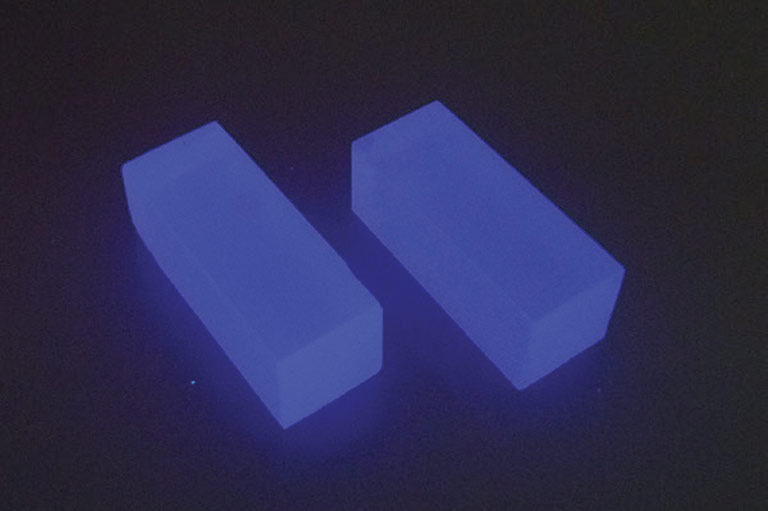
CLYC scintillator crystals under UV excitation
Media Credit: Radiation Monitoring Devices, Inc.
Knowing how the CLYC scintillator mixture behaves and solidifies in microgravity will help Churilov and RMD determine the importance of certain aspects of their production process on Earth. The SUBSA experiments will enable RMD to observe whether convection limits the size and physical quality of CLYC scintillator crystals for improved radiation detection.
“When you eliminate convection, other subtle processes can be directly observed,” Churilovsaid. “This will give us immediate guidance of how significant reducing convection in the melt is for our crystal growth.”
LITTLE FURNACE, BIG BENEFITS
The SUBSA furnace is a relatively small facility, but the advancement in fundamental knowledge about the formation and purity of semiconductor and scintillator crystals may have dramatic impacts in the development of next-generation radiation detectors. Although manufacturing these materials in space may not yet be economically feasible, the knowledge gained by the SUBSA studies will accelerate progress in ground-based production.
“The purpose of our microgravity experiment is not necessarily to grow better crystals in space,” Churilov said. “Our ultimate goal is really to understand the formation of defects in these crystals and apply that to our production process back on Earth.”
However, in-orbit manufacturing of materials may not be as far off as one might think. Various companies are currently exploring the possibility of space-based platforms for producing consumer products or commercially important materials. The commercialization of low Earth orbit(Abbreviation: LEO) The orbit around the Earth that extends up to an altitude of 2,000 km (1,200 miles) from Earth’s surface. The International Space Station’s orbit is in LEO, at an altitude of approximately 250 miles. may include steps toward such next-generation space stations, and current payloads on the ISS National Lab include proof-of-concept initiatives in this realm. But for Ostrogorsky and Churilov, the advancement of knowledge is enough for now.
“Trying to think that someday we are going to have crystal production in space is too ambitious, too far away,” Ostrogorsky said. “The idea is to do the experiments and get new knowledge so we can do some work on Earth.”