Crystal Clear: Super-Sized Protein Crystals From Space Could Help Treat Diseases on Earth
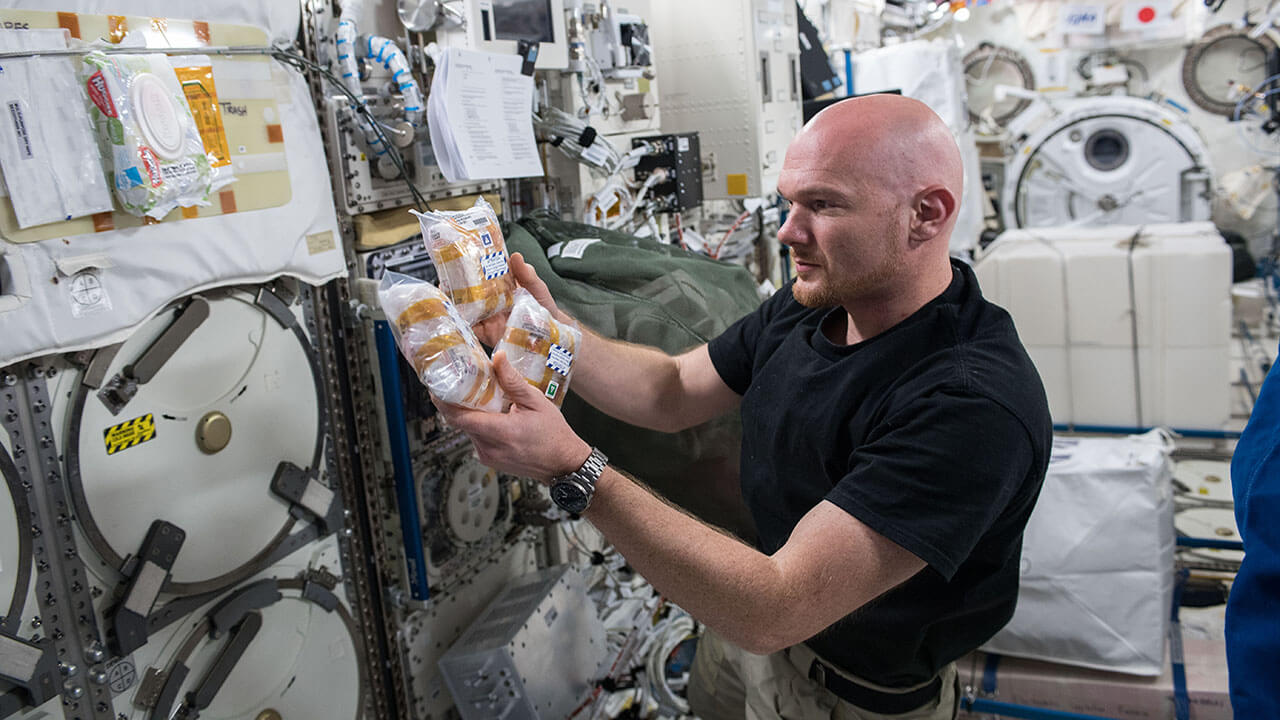
European Space Agency astronaut Alexander Gerst places Toledo Crystallization Boxes (TCBs) into storage onboard the ISS.
Media Credit: NASA
July 26, 2022 • By Stephenie Livingston, Staff Writer
In Greek mythology, Pandora’s Box releases disease and other miseries into the world. But in real life, a very different type of box uses microgravityThe condition of perceived weightlessness created when an object is in free fall, for example when an object is in orbital motion. Microgravity alters many observable phenomena within the physical and life sciences, allowing scientists to study things in ways not possible on Earth. The International Space Station provides access to a persistent microgravity environment. to do the opposite: fight disease back on Earth.
University of Toledo biochemist Timothy Mueser’s “Toledo Crystallization Box” utilizes the microgravity environment of the International Space Station (ISSInternational Space Station) to change the physical form of proteins involved in human disease into large crystals. Such crystals can provide new data about the physical structure of proteins that can offer insights into their function—information essential to developing new pharmaceutical drugs to help patients.
Using protein crystals grown in the Toledo Crystallization Box during an ISS National Laboratory-sponsored investigation, Mueser and his colleagues did something that had never been achieved on Earth. Using neutron diffraction, the team pieced together the structure of bacteria-derived tryptophan synthase (TS), a key enzyme involved in the ability of salmonella and other bacteria to cause infection. This represents the first time researchers have directly visualized the position of protons within vital functional elements of the enzyme’s structure, allowing Mueser’s team to develop physical models of the enzyme that are more accurate than ever before.
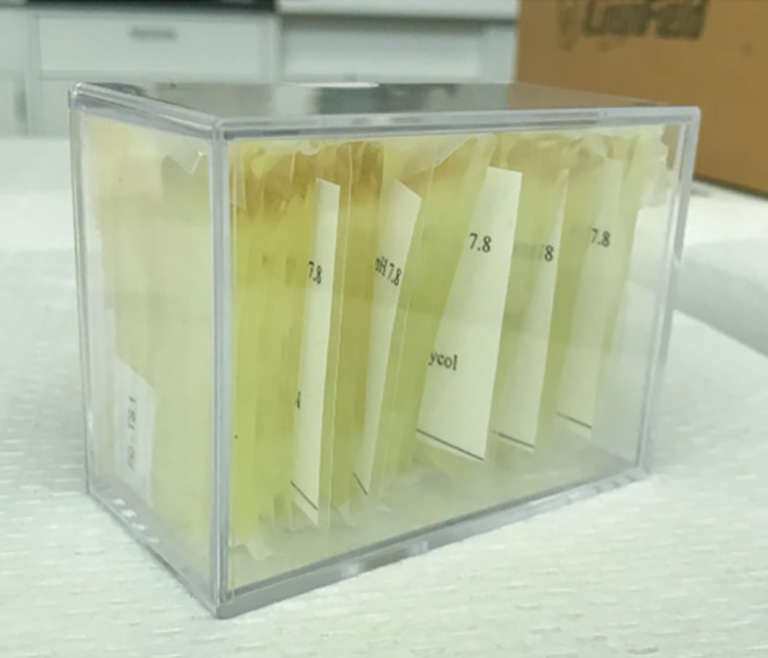
A filled Toledo Crystallization Box containing 50 individual crystallization experiments.
Media Credit: Image courtesy of npj Microgravity
Knowledge of this atomic-level structure aids in developing antibiotics that better target and inhibit bacterial pathogens, said Mueser, a professor of chemistry and biochemistry at the University of Toledo’s College of Natural Sciences and Mathematics. His team’s space-based experiment is detailed in a study recently published in the journal npj Microgravity.
To achieve the neutron structure for TS, microgravity was vital. On Earth, some proteins crystallize with inclusions and high mosaicity (misalignment within the crystal lattice during the crystal’s growth) that create imperfections in the physical image of the structure. However, in microgravity, crystals form in a sort of slow-motion—the absence of gravity-driven convection allows proteins to crystalize slowly and often more orderly. For some proteins, like TS, this results in bigger crystals than can be achieved on Earth.
“The crystals were beautiful—we got exactly what we were after,” Mueser said. “We had no way of getting those crystals without that microgravity flight.”
Microgravity as a Magnifying Glass
In the same way that we use visible light captured by cameras to observe details in pictures, scientists observe protein crystals at an atomic level by using X-ray or neutron diffraction to pinpoint the physical position of atoms in the protein. Such structural data can be used to uncover new targets for drug development. Traditional structure-based drug design uses 3D models created via X-ray diffraction, but these models sometimes have incomplete structural data or lack detail. Neutron diffraction, unlike X-ray diffraction, reveals the position of hydrogen atoms, which make up around 50 percent of the atoms in proteins and are missed by X-ray diffraction.
The public Protein Data Bank holds more than 100,000 protein structures, but less than 100 have neutron diffraction data. When drugs are designed against incomplete protein structures, the wrong targets can be identified, leading to serious side effects.
The challenge is that for neutron diffraction to work, you need crystals that are well-ordered and large—about 100 times bigger than the crystals typically used for X-ray diffraction. This can be difficult to achieve on Earth, as it can take years to identify the best conditions for the crystals to grow, and the crystal quality may not be high enough for neutron diffraction. Microgravity provides a solution for quickly growing large, high-quality crystals for some proteins. For this reason, Mueser and his team took their protein crystallization to space.
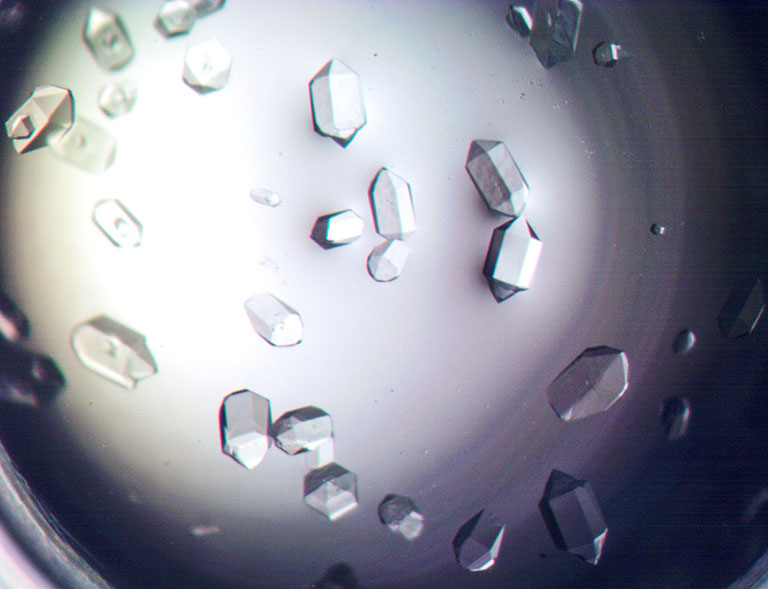
Aeropyrum pernix Flap Endonuclease-1 (FEN-1) protein crystals grown under Earth gravity conditions are shown. While impressive, these crystals from a past experiment by Timothy Mueser’s lab are smaller and of less quality than those his team grew during their most recent experiments onboard the ISS.
Media Credit: Image courtesy of University of Toledo
Mueser’s 2019 ISS investigation is part of an ongoing evolution of molecular crystallization studies using microgravity that spans decades. “It’s pretty exciting,” Mueser said. “This work really started with the flight of the first protein crystallization experiment way back in the day.”
Probing Proteins for Targeted Drug Development
When the team’s experiment launched on SpaceX’s 18th Commercial Resupply Services (CRS) mission, it contained three enzymes (proteins that act as catalysts in biological processes) specifically picked for their dependence upon vitamin B6-derived pyridoxal 5′-phosphate (PLP). The three were: Salmonella typhimurium tryptophan synthase (a complex of tryptophan synthase from the bacterial pathogen salmonella), another protein related to heart and liver disease, and a complex of two proteins involved in DNA repair.
Mueser’s team is interested in PLP-dependent enzymes because they are common to many organisms in all branches of life and are part of a diverse family of enzymes responsible for more than 100 different chemical reactions in cells. Despite their prevalence and importance, the relationship between the structure and function of the enzymes is poorly understood. Researchers need to see the enzymes in their entirety on an atomic level to complete their structure and understand how they function.
Because bacterial-derived TS is involved in the metabolic processes of many different microorganisms, it is a model enzyme for understanding PLP-dependent enzymes. This, coupled with the fact that TS does not occur naturally in humans, makes it a common target for drugs designed to inhibit human pathogens that depend on it, including infections caused by salmonella and staphylococcus (staph infection).
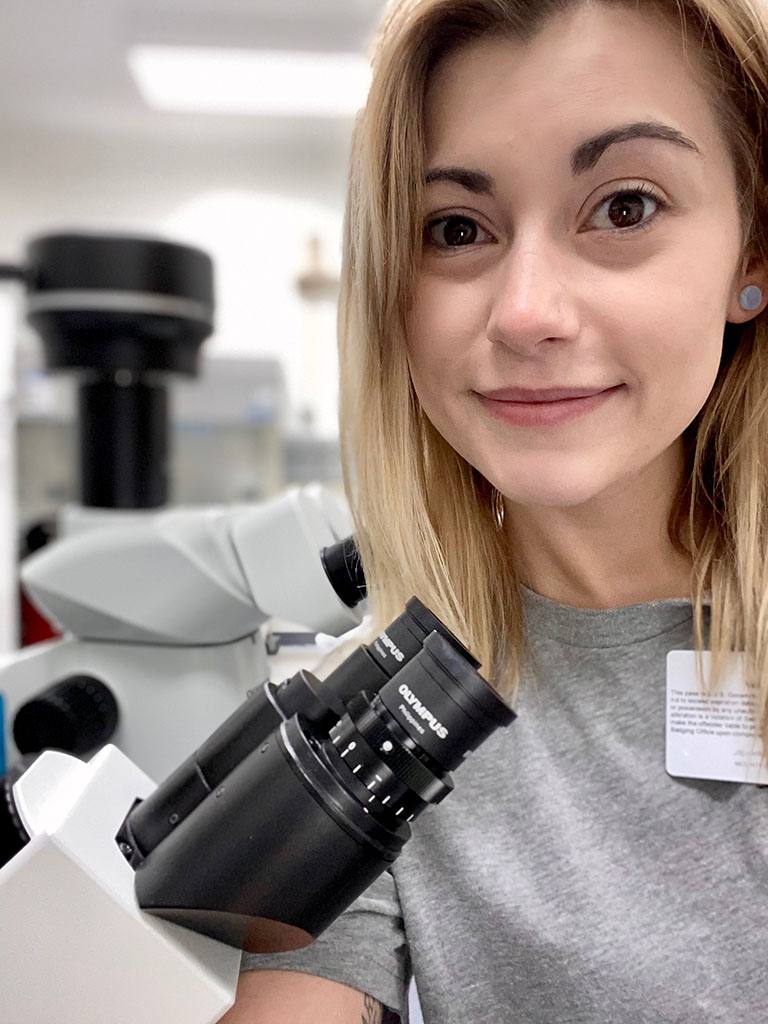
Biochemist Victoria Drago examines protein crystals under a microscope.
Media Credit: Image courtesy of Victoria Drago
“While our team doesn’t focus on eliminating salmonella, we want to figure out how we can intervene via PLP-dependent enzymes,” said biochemist Victoria Drago, a recent Ph.D. recipient from Mueser’s lab who led the team’s investigation on the ISS. “Our primary goal is to understand PLP and how it is modulated by PLP-dependent enzymes to be so versatile. ”
If you do not understand the action occurring inside the enzyme, it is difficult to inhibit it, Drago explained. In an infection propelled by PLP-dependent enzymes, one of the steps involves the enzyme’s hydrogen atoms being shuffled around during a reaction. Thus, understanding where the hydrogen atoms are and how they move inside the enzyme is essential to designing targeted antibiotics, she said.
A complete neutron structure for TS could lead to better-targeted drugs to treat infections driven by PLP-dependent enzymes, such as salmonella infection, which affects about 1.35 million people in the United States each year. “It is possible salmonella could be thwarted in its growth if you could reduce its ability to get tryptophan, resulting in less severe illness or no illness at all, ” Drago said.
Neutron Diffraction in Pandemic Times
The team’s ISS investigation consisted of three Toledo Crystallization Boxes—one for each enzyme. Inside each box sat 45 to 50 quartz capillaries containing protein solutions, sealed on one end with beeswax and the other with a permeable membrane. These capillaries were put inside a small bag that included a crystallization solution that slowly diffuses over several months to form crystals. After launching in 2019, the boxes sat tightly packed and untouched in a locker on the ISS for six months while the crystals formed.
The investigation returned to Earth on SpaceX CRS-19 in January 2020 amid a looming global pandemic. Mueser and the team were eager to assess the quality of the crystals and were a little nervous. They knew things go wrong in experimentation. Sometimes protein crystals that are hard to grow in gravity conditions also fail to grow in microgravity. Capillaries can break. Protein solutions can go wrong.
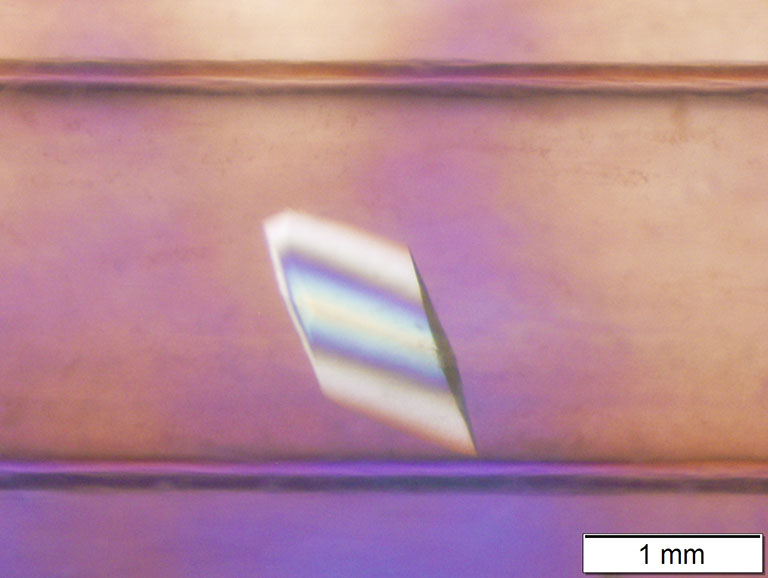
Microgravity-grown crystal of perdeuterated tryptophan synthase (TS) for neutron diffraction.
Media Credit: Image courtesy of Victoria Drago
After the capsule splashed down in the Pacific Ocean and samples were shipped to Texas, Mueser picked up the experiment at NASA’s Johnson Space Center in Houston. When he opened the Toledo Crystallization Boxes, he found more than 150 crystals distributed among the capillaries in the three boxes. Crystals of aspartate aminotransferase—a protein related to heart and liver disease—in the first box were the perfect size for the team’s planned X-ray diffraction studies. The second box contained two DNA repair proteins that form a transient complex, but only remnants of crystals were found. For these two proteins, it is not easy to grow large crystals on Earth or in space. However, the crystals in the third box, which contained deuterated TS, were different. In that box, there were near-perfect, super-sized (>1 mm3) crystals. The team was thrilled.
Mueser flew with some of the crystals to the Oak Ridge National Laboratory in Tennessee, where the Spallation Neutron Source (SNS) facility and the High Flux Isotope Reactor (HFIR) are maintained by the U.S. Department of Energy. The HFIR facility allowed the team to begin their data collection using neutron diffraction, although Mueser saved the largest, highest-quality crystals for neutron diffraction at Institute Laue-Langevin in Grenoble, France. This lab houses another nuclear reactor, and his colleagues there had expressed the protein in heavy water to make it more amenable to neutron diffraction studies. However, by this time, the COVID-19 pandemic was well underway, and travel restrictions prevented him from delivering the crystals in person as he typically would. So, Mueser decided to take a risk.
He again tightly packed the hard-earned crystals in bubble wrap and tape and placed them in a box—but this time, it was a FedEx box. The experiments had traveled almost 50 million miles in low Earth orbit(Abbreviation: LEO) The orbit around the Earth that extends up to an altitude of 2,000 km (1,200 miles) from Earth’s surface. The International Space Station’s orbit is in LEO, at an altitude of approximately 250 miles., but the last 3,000 miles back on the ground would be the most precarious.
Luckily, the crystals made it to France intact. But due to delays caused by the pandemic, Muesser’s colleagues in the hard-hit South of France took more than a year to finish neutron diffraction and data collection for the TS crystals. As data finally poured in from Grenoble, Drago began piecing together the atomic-level images to form the first neutron structure of TS. “For the first time, it’s all there for your eyes to see,” said Drago.
She is studying the structure and developing more accurate models for understanding how PLP influences the enzyme’s reaction and role in salmonella and other pathogens. These structural models may also be used more broadly for the general study of PLP-dependent enzymes because TS is a model for many of them.
Designing the Toledo Crystallization Box
Several devices for growing protein crystals have flown in space since the 1980s, but they were for short-duration experiments and were not designed to grow large crystals for neutron diffraction. Over the past 15 years, access to long-term microgravity on the ISS has led to advancements in this type of space-based crystal growth. Additionally, as the availability of neutron beams at research centers emerged, so has a new priority among molecular crystallization researchers to develop devices made explicitly for growing large crystals for neutron diffraction.
Early Space-Based Crystallization for Neutron Diffraction
The first investigators to grow neutron diffraction-quality crystals using the GCB on the ISS were from the University of Alabama and included Joseph Ng, a biochemistry professor at the University of Alabama in Huntsville. Ng said the Toledo Crystallization Box is a suitable replacement for the GCB to grow crystals big enough for neutron diffraction by counter-diffusion equilibration. Insight gained from the analysis of such microgravity-grown crystals could lead to valuable advancements in drug design.
“Three-dimensional structures of proteins deciphered by neutron crystallography can provide atomic details that cannot be obtained by X-ray crystallography alone,” Ng said. “The accuracy of knowing the atomic spatial arrangement is critical for structure-based drug discovery processes.”
Mueser designed the Toledo Crystallization Box to perform a very slow, diffusion-controlled crystallization in microgravity. His team based the design on a previous NASA-approved device, the “Granada Crystallization Box” (GCB) developed and sold by Triana Science and Technology in Granada, Spain for salt-based counter-diffusion. Mueser and his team named their new device the “Toledo Crystallization Box” (TCB) in homage to the GCB. However, the Toledo Crystallization Box is different because it allows broader crystallization conditions and has a more cost-effective off-the-shelf design.
“Our box is simple,” Mueser said. “It’s about $100 worth of material wrapped in bubble wrap and mylar tape.”
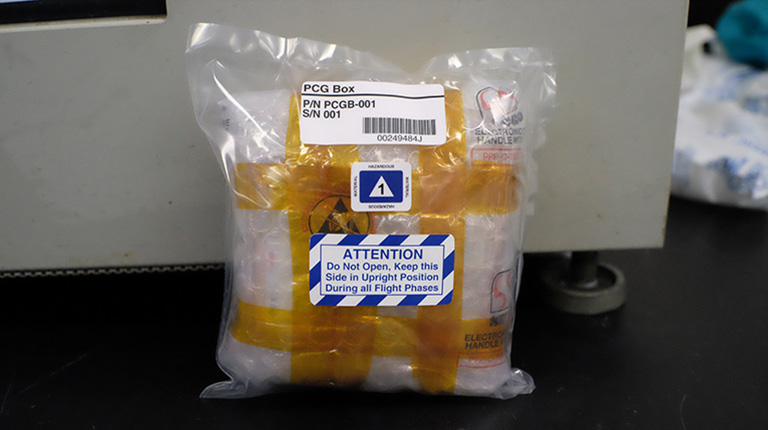
The Toledo Crystallization Box (TCB) shown here is packed with 50 individual crystallization experiments and ready for flight.
Media Credit: Image courtesy of Victoria Drago
The Toledo Crystallization Box has been tested twice on the ISS, most recently in the team’s 2019 investigation, and the resulting TS crystals demonstrated that it can successfully yield “beautiful, super-sized” crystals for neutron diffraction, Mueser said. Now that its success has been demonstrated, the team plans to use the Toledo Crystallization Box for future ISS experiments, including those by other labs. Drago said, “Sending our device back to the ISS in the future, packed with our experiments and experiments from others, is definitely something we hope for and that we’re working toward.”
For Mueser, the path forward is a progression of his life’s work. “As a young scientist back in the 1990s, I worked with the scientists that solved the first structures of TS,” he said. “Now, decades later, we’re moving forward with the first neutron diffraction data for TS to do more experiments as we decipher how this vitamin (PLP) works. The number of possible things we could do from here is limitless—it’s pretty exciting.”