A Small Drop With a Big Impact: Fundamental Science in Space to Improve Medicine on Earth
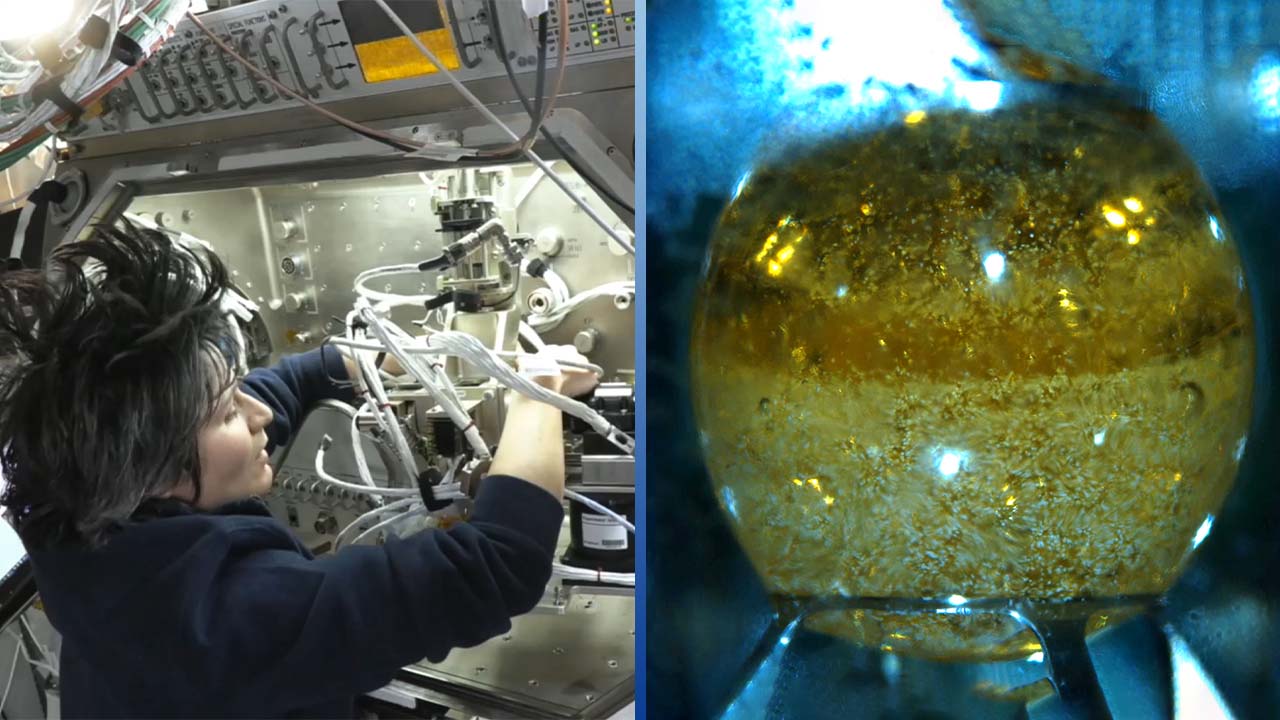
ESA astronaut Lt. Samantha Cristoforetti installing the Ring Sheared Drop system into the Microgravity Science Glovebox on the ISS (left). The Ring Sheared Drop system containing a concentrated solution of human serum albumin (the main protein constituent of blood); the small white dots are glass tracer particles that allow researchers to study fluid flow of biofluids in space (right).
Media Credit: NASA (left)/Joe Adam (right)
September 29, 2023 • By Amelia Williamson Smith, Upward Managing Editor and ISS National Lab Science Communications Manager
It’s 10 p.m., and researchers and students excitedly gather inside a lab at Rensselaer Polytechnic Institute (RPI) in New York. All are focused on the computer screen, looking with fascination as a colorful one-inch sphere of protein solution is deployed into the Ring Sheared Drop system on the International Space Station (ISSInternational Space Station). The liquid sphere, pinned between two rings, looks like a beautiful spinning marble and is mesmerizing to watch. Even more intriguing is that studying this drop of protein solution in space could be key to improving pharmaceutical manufacturing on Earth.
The first protein-based therapeutic, insulin, was approved in 1982. Since then, this new class of drugs has become critical in treating and preventing diseases that range from cancer to HIV. There are hundreds of protein therapeutics on the market, with many more in clinical trials. In 2020, the global protein therapeutics market was valued at more than $280 billion and is estimated to reach more than $565 billion by 2030, according to Allied Market Research.
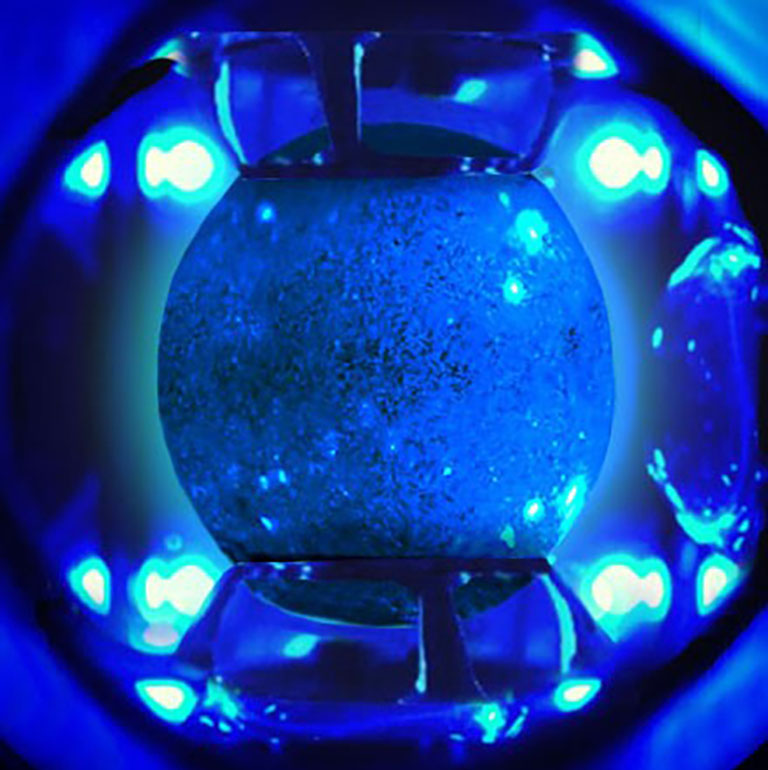
Human serum albumin drop under blue illumination in the Ring Sheared Drop system on the ISS.
Media Credit: Rensselaer Polytechnic Institute
However, there is an Achilles’ heel in protein therapeutic manufacturing, explained Amir Hirsa, professor of mechanical, aerospace and nuclear engineering at RPI. During manufacturing, proteins can aggregate (clump together), negatively affecting drug quality and reducing the amount of viable medication or vaccine produced.
If scientists better understood the complex motion of proteins in solution and what leads to aggregation, they could look for ways to avoid or reverse it, which would be invaluable to the field of medicine. An added complexity to ground-based studies is that protein solutions must be put into a beaker or other container, and the container walls alter how the proteins behave. This makes observing various aspects of protein flow and behavior challenging.
To get past this obstacle, a team of researchers from Arizona State University and RPI turned to the ISS National Laboratory®. In an investigation funded by the U.S. National Science Foundation, the team leveraged microgravityThe condition of perceived weightlessness created when an object is in free fall, for example when an object is in orbital motion. Microgravity alters many observable phenomena within the physical and life sciences, allowing scientists to study things in ways not possible on Earth. The International Space Station provides access to a persistent microgravity environment. to do something that cannot be done in ground-based labs—study a drop of liquid protein without a container. In space, liquids don’t fall. Instead, they form into self-contained, floating spheres, making containers unnecessary.
“In microgravity, the experiment’s uniqueness is the fact that it’s essentially containerless, so the interaction of protein with solid walls is not a dominant effect as it would be on Earth,” Hirsa said. “Having a containerless biochemical reactor at the 10-milliliter scale is the selling point. Without microgravity, this wouldn’t be possible.”
The Problem With Proteins
Proteins are large, complex molecules made of long chains of amino acids, and a protein’s structure (how it folds) determines its function. But proteins are flexible and can change how they fold depending on their environment, complicating pharmaceutical manufacturing. For example, some proteins change structure when they come into contact with air at the surface of a liquid. This is because some sections of proteins are attracted to water, while others are repelled by water, explained Juan Lopez, a professor at Arizona State University.
“When a protein is in solution completely, the part that doesn’t like water gets folded in and protected by the parts that do like water,” Lopez said. “Then, when the protein comes to the surface, the part that doesn’t like being in water can pop out, and once it does that, it can start linking up with other proteins.”
Video Credit: Joe Adam
As the proteins link together, they form a film on the surface of the fluid that behaves differently than the protein solution below. As the film interacts with the rest of the fluid, it changes the behavior of the whole solution, which is problematic for therapeutics. During pharmaceutical manufacturing, protein solutions are exposed to air at several points, so understanding how proteins react when they meet air is crucial.
Another environmental factor that can change a protein’s structure is stress from shear, which arises from the force produced when fluids slide past each other. During pharmaceutical manufacturing, protein solutions are mixed in bioreactors that contain hundreds or thousands of liters of solution. The mixing process induces shear in the liquid, and manufacturers must be careful not to mix the solution too quickly or too hard because the stress can alter protein structure, Hirsa explained.
Shear stress can also affect flow in protein therapeutics. The reason has to do with the fact that the protein solutions are highly concentrated. It is impractical to put a large amount of fluid into a patient to provide the right dose. So, the protein solution is concentrated into a smaller amount of fluid that can be given as an injection or through an IV. But when there is a high concentration of proteins, it alters the fluid properties of the solution.
Normally, shear stress does not change the viscosity (resistance to flow) of a fluid. Think of stirring a glass of iced tea. The viscosity, or thickness, of the tea remains the same no matter how hard you stir it. This is called “Newtonian” fluid behavior because the fluid follows Newton’s law of viscosity. But concentrated protein solutions do not behave this way. In such “non-Newtonian” fluids, shear stress changes the fluid’s viscosity, and it thins or thickens, affecting its flow.
“Ketchup is a great example of a non-Newtonian fluid,” Hirsa said. “If you put ketchup on your turkey burger, you want it to stay there and not run off, but you also want the ketchup to come out of the bottle easily when you squeeze it.”
When you apply shear stress to the ketchup by squeezing the bottle, the ketchup becomes less viscous and flows out more easily. When the shearing stops, the ketchup becomes more viscous again. That’s an example of shear thinning, and some proteins react similarly. But other proteins do the opposite, Hirsa explained.
“Shearing can make the protein solution more viscous and turn it into a gel and eventually a solid,” he said, “and these complications are headaches in pharmaceutical manufacturing.”
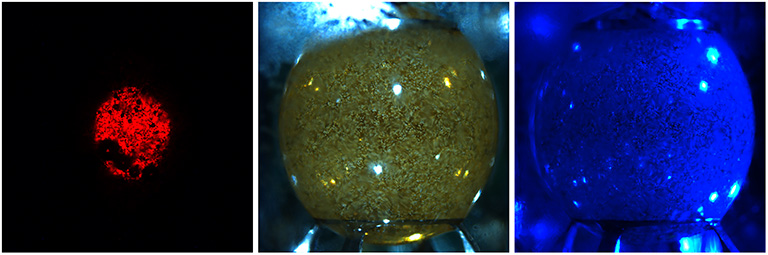
The Ring Sheared Drop system on the ISS containing a concentrated solution of human serum albumin, the main protein constituent of blood. Small white dots are glass tracer particles that allow researchers to study fluid flow of biofluids in space.
Media Credit: Joe Adam
To alleviate the headache, scientists need to understand how protein behavior changes at air-liquid interfaces and how the altered proteins interact with other non-Newtonian flow phenomena in the fluid below the surface. With this information, researchers could create robust models to predict protein behavior in specific situations, which would be extremely beneficial to the field of medicine.
“Trying to understand what’s happening on the surface of liquids is becoming more and more relevant to pharmaceutical applications—trying to figure out at what stage non-Newtonian behavior kicks in at the interface and in the bulk fluid, as well as the two-way coupling between the two,” Hirsa said. “They’re not independent and are touching each other, so their interaction needs to be understood, and we expect our results to contribute to that.”
Going Containerless in Space
To study protein solution interactions without effects from solid walls getting in the way, Hirsa and the team launched an investigation to the ISS. Here, they could conduct experiments using the containerless Ring Sheared Drop system designed, developed, and operated by ISS National Lab Implementation Partner(Abbreviation: IP) Commercial companies that work with the ISS National Lab to provide services related to payload development, including the translation of ground-based science to a space-based platform. Teledyne Brown Engineering in tandem with the RPI research team.
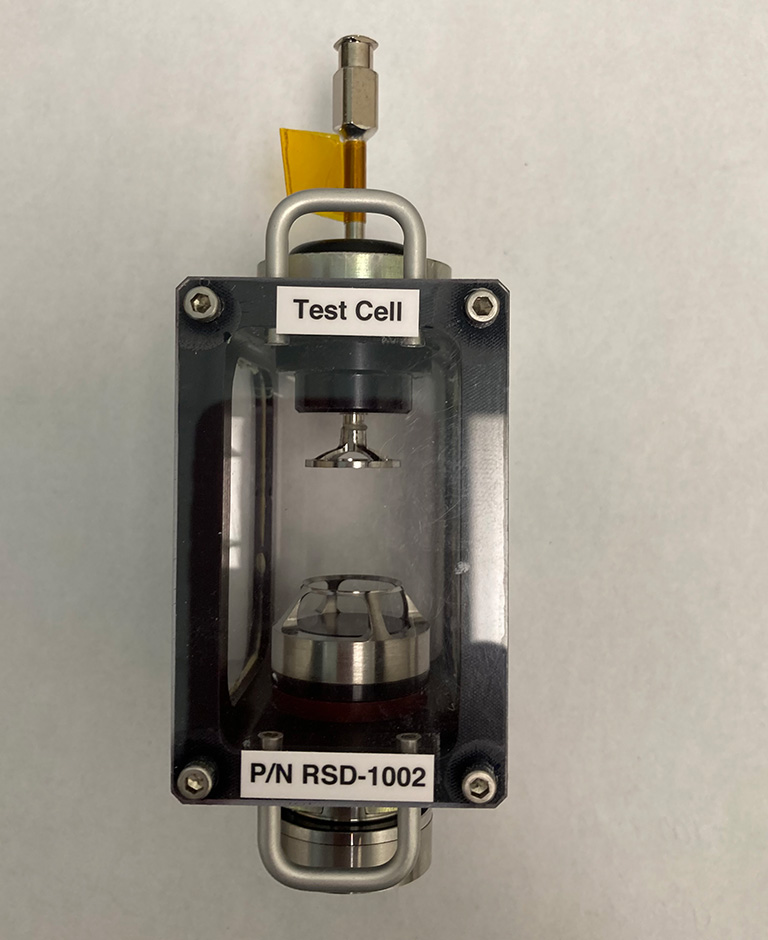
A drop deployment test cell for the Ring Sheared Drop system on the ISS.
Media Credit: NASANational Aeronautics and Space Administration
The system consists of a small apparatus that houses a test cell containing an injection tube and two rings. Syringes of protein solution are installed into the system, and drops are deployed through the injection tube that extends into the center of one of the rings. As the self-contained drop grows in diameter to a size of one inch, it eventually contacts the second ring on the other side and is pinned between the two rings. Then, one ring can be rotated while the other remains stationary to create rotation and shear within the drop. Video and still cameras in the system capture imagery of the drop, and a microscope allows researchers to get a closer look at the drop’s interior. The system can also visualize the drops with red, blue, or white LED lighting, enabling the measurement of different fluid properties in the drop.
“The critical part of the Ring Sheared Drop system is the ability to observe these deployed drops in real time and induce shear into the drop by spinning the lower ring,” said Paul Galloway, who recently retired from his position as senior systems engineer at Teledyne Brown Engineering. “The shear induces flow within the drop, and we use the cameras to view the flow field.”
For this investigation, the team used two proteins: human serum albumin (HSA) and bovine serum albumin (BSA), which make up the majority of protein in the blood of humans and cattle, respectively. The team chose these proteins because they are very accessible and well-studied. Also, HSA and BSA are similar in structure and behavior to many other proteins, explained Joe Adam, a postdoctoral researcher at RPI who was on the research team.
“The idea is to use very approachable proteins like HSA and BSA and determine their overall function and motion methodologies in microgravity and on Earth, and then try to extend findings to other proteins,” Adam said. “If you have a model that’s working for a very well-known and well-studied protein, you can start to test your theory, hypotheses, and rules governing equations for motion with other proteins and refine these methods for whatever application you’re looking for.”
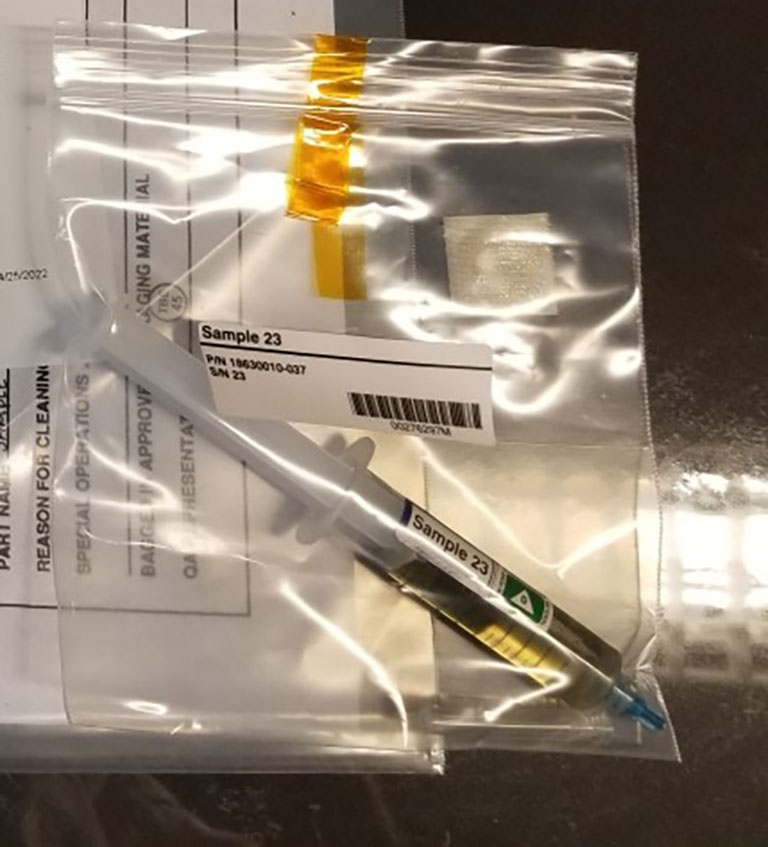
Protein solution sample for the investigation loaded into a syringe for launch to the ISS.
Media Credit: Teledyne Brown Engineering
Teledyne Brown Engineering sent the research team a syringe kit with approved NASA labels to prepare the experiment. All the researchers had to do was fill the syringes with the HSA and BSA protein solutions—which they had prepared and tested for quality assurance—and freeze them. Then, Teledyne Brown Engineering took care of all the logistics to keep the samples frozen and ensure they arrived at Kennedy Space Center and got through the preparation process for launch, said Galloway, who has performed the systems engineering and system safety function for the Ring Sheared Drop system since it was in the early concept phase.
“The real selling point of the Ring Sheared Drop system is the simple and fast integration process that we can use to get your science onboard the space station,” Galloway said. “The research team just provides the flight-approved liquid protein solution along with a plan and timeline to complete their investigation on station. They don’t have to build anything at all, and that’s the real beauty of Ring Sheared Drop as a system for science users.”
Interactions In Orbit
Once the experiment was on station, the team deployed drops of the two proteins at three concentration levels. One was the level naturally found in the body (40 milligrams per milliliter). Another was a highly concentrated solution like that of pharmaceutical applications (10 times the level found in the body). The last was a diluted solution like that used in biotechnology sensing applications (one-tenth the body’s level).
The team observed the drops at several time points: during drop formation and pinning, as shearing began, during steady shearing, and as shearing stopped and the drop returned to an equilibrium state. The researchers also examined the fluid’s turbidity, or cloudiness, and used small hollow glass tracer particles in the drop to track the velocity of the flow.
“These particles move, and their motion can be detected on a large scale in imagery,” Adam said. “That can tell us a lot about the motion of the fluid and its behavior.”
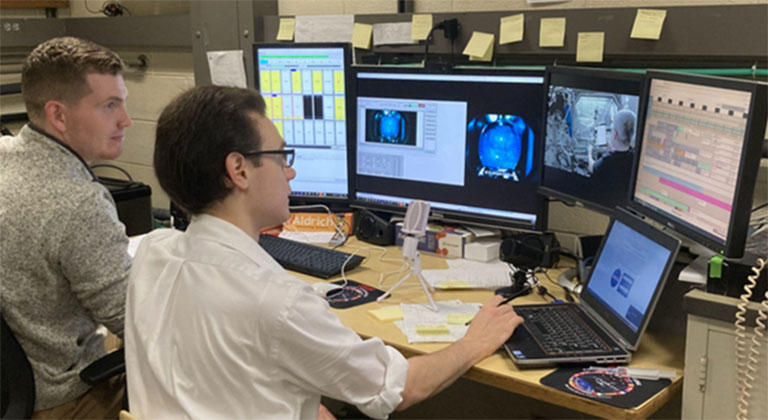
RPI postdoctoral researchers Joe Adam (right) and Partrick McMackin (left) commanding the investigation on station from their lab at RPI.
Media Credit: Rensselaer Polytechnic Institute
While the experiment was being done on station, the team watched on their computers in real time, with a view of the astronaut performing the test cell installation (and eventual removal) and a view of the drops inside the Ring Sheared Drop system. If a protocol was not working as planned and a change needed to be made, the researchers could alert the astronaut. At one point, the system’s motor was malfunctioning, and the team helped determine that a screw had come loose and put together a protocol for the astronaut to repair the system.
“It’s not just watching our experiment work. It’s being able to step in and say, ‘don’t turn it that way,’ or ‘try this if that’s not working,’” Hirsa said. “Having the ability to watch in real time what’s going on and be involved in designing the protocol to fix the issue is priceless.”
From Fundamental Science to Application
Now that the experiment on station is complete, the team is analyzing the data collected, and several non-Newtonian flow models are being implemented and tested against the data. “All the heavy lifting on the analysis and modeling will happen in the coming months,” Hirsa said. “We’re beginning to digest the data and expect to have a publication before the end of the year.”
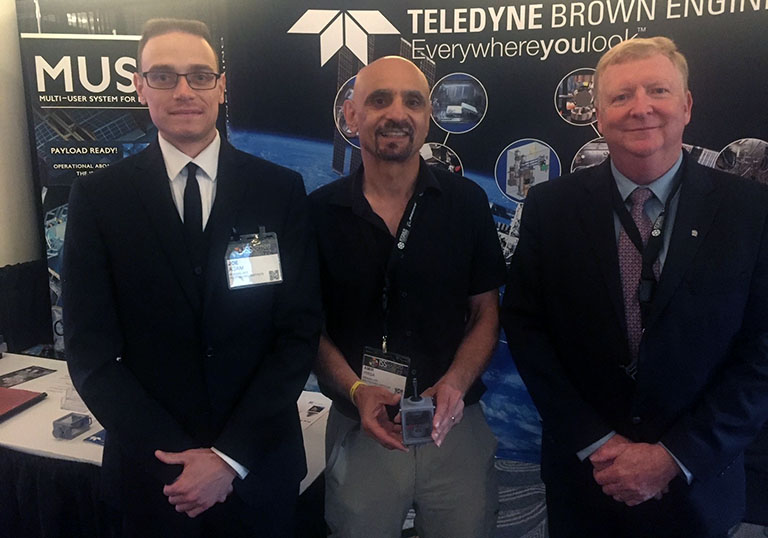
Paul Galloway from Teledyne Brown Engineering (right) with RPI researchers Joe Adam (left) and Amir Hirsa (middle), who is holding a Ring Sheared Drop system test cell, at the 2022 ISS Research and Development Conference.
Media Credit: Paul Galloway
The team hopes to use fundamental knowledge gained from the investigation to improve predictive models and better understand the factors that lead to protein aggregation in highly concentrated solutions. “At the fundamental level, understanding how proteins denature at an interface, their general flow, and mechanics is required for developing applied knowledge,” Adam said. “We can then determine, is this reversible? Can you use another fluid mixing process to reverse the state of the proteins and correct them?” Being able to control or reverse protein aggregation in therapeutic manufacturing would be a game-changer in the medical industry.
Thinking back to the lab full of students watching in captivation as the ISS crew worked on the experiment, Hirsa says there is a second aspect to the project’s success—the impact it is having on the future science, technology, engineering, and mathematics (STEM) workforce. Throughout the project, the team alerted students when drop deployment was happening on station so they could come to the lab to watch and get involved. The students of today will become the workforce of tomorrow, and engaging students in meaningful, exciting science is key to inspiring them to pursue careers in STEM fields.
“I can draw top-notch students to my lab because they come and see the excitement as undergrads and stay to get a graduate degree and go on as a postdoc,” Hirsa said. “That excitement, the draw of space—especially watching an experiment on the ISS—is fantastic.”